Cells maintain their health and avoid disease by proper regulation of biochemical activities and signaling pathways. For example, utilization and appropriate storage of glucose will be regulated by the available energy in the form of ATP, and regulation of kinases are needed to coordinate cellular responses to existing conditions and environmental stimuli. A primary means of regulation of signaling proteins, receptors, and enzymes is through allosteric regulation. Allosteric regulation occurs when a ‘signaling’ molecule (e.g. a metabolite or another protein) binds to a protein at one site of its structure and alters the activity of that protein at a different location. As common as this is throughout biology, how this long-range communication occurs in a physical mechanism is poorly understood, even in 2024 amidst a massive amount of high-resolution protein structures.
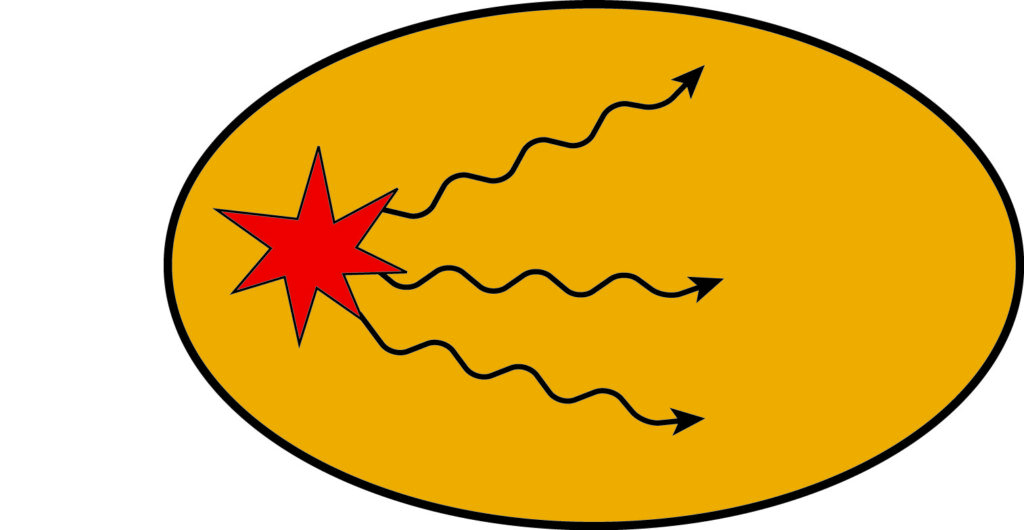
The promise of a better understanding of allostery in proteins lies in the discovery of allosteric drugs, as well as in the design of allosteric proteins. In order to pursue these goals, gaining a deeper understanding of allosteric mechanisms will be helpful. We believe that a key reason why allosteric mechanisms are not well understood is because in many cases allostery is enabled by protein dynamics. The dynamics of proteins are typically not evident in their structures, and hence characterization requires direct probing of dynamics, as with NMR relaxation methods.
Our understanding of protein allostery begins with the original models of allostery, namely the MWC and KNF models from the 1960s. These models were proposed in the context of symmetric, multisubunit enzymes, in which subunits can adopt a more active (R for “relaxed”) or less active (T for “tense”) conformation. The MWC model imposes full subunit symmetry, leading to only two (R and T) states and “concerted” conformational switching between them. The KNF model allows individual subunits to switch and hence is “sequential” in nature. The binary nature of these models, and the lack of any interior information finer than R and T, leaves these models bereft of conformational intermediates or protein dynamics in general (except for the R-T conformational switch). A primary goal of our research is to enhance our understanding of allostery by gaining interior information beyond a global (two-state) conformational change, and to challenge the simplicity of the historical models for applicability to allostery in monomers.
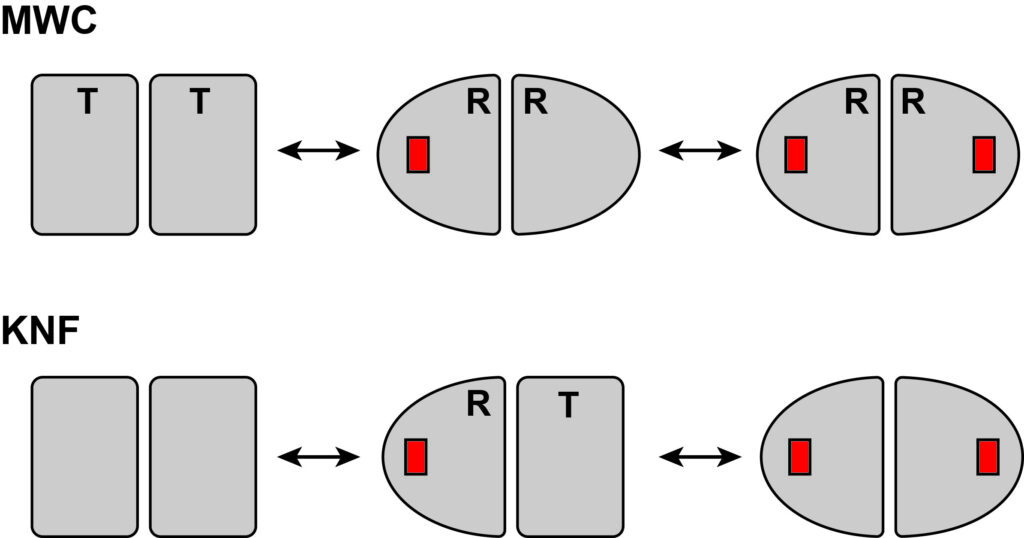
Studies over the past two decades indicate that protein dynamics are likely playing an important role in allosteric function. It is likely a combination of structural and dynamic changes that enable a localized binding event or chemical modification (e.g., phosphorylation) to propagate its effects over long distances. NMR spectroscopy makes clear how even a single mutation (or bound ligand) can affect many signals in its spectrum (HSQC overlay below). We view the combination of NMR and experiments on systems designed to link dynamics (or structure) with function as an approach that will yield important new insights into mechanisms of allostery.
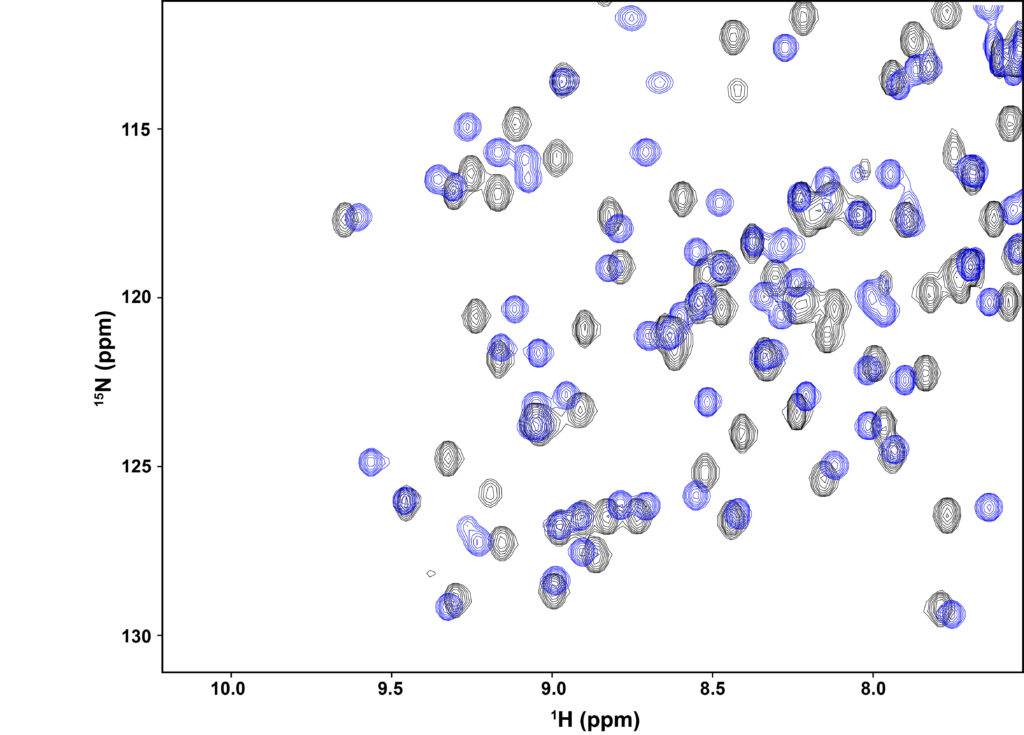
Overlay of 2D 1H-15N HSQC spectra from the second PDZ domain (“PDZ2”) from hPTP1E. The blue contours (PDZ2 bound to short peptide) show extensive shifting of most peaks from the black contours (unbound PDZ2). The shifting extends well beyond the binding site, showing how most of the protein “senses” ligand binding, even though PDZ2’s conformation is not changed. See Fuentes et al. (PMID 14698303) and Zhang et al. (PMID 20839809).
Allosteric function is found well beyond the classical, multisubunit enzymes highlighted in the past. Examples include monomeric proteins such as kinases, transcription factors (DNA binding proteins), and the small signaling proteins called GTPases, to name a few. Enzymes in general can often be allosterically regulated. Another major class of allosteric proteins are G-Protein Coupled Receptors (GPCRs), which bind molecules on the extracellular side of the membrane and transmit this to functional changes on the intracellular side. To generalize, anytime the cell requires some sort of coordination of protein function, allostery is very likely going to be behind this. In other words, allostery is pretty much everywhere.
Despite clear allostery in the above-mentioned systems, dynamics can be challenging to characterize in many of those systems. Thus, we choose to study systems that show clear evidence of allosteric function but are also highly amenable to NMR studies, such as chorismate mutase, thymidylate synthase, and an engineered optogenetic GTPase (Cdc42, see Jain et al., PMID 36972433).
What is allostery?
From our reading of the literature, we find that the term “allostery” or “allosteric” is used differently by different authors and groups. We adopt the more stringent interpretation, in which allostery is the non-zero effect that ligand binding (or chemical modification) at a distal site has on some kind of activity, such ligand binding or catalytic function, at the active site. Sometimes others use “allostery” to mean simply that there is a conformational change that takes place, but in our opinion this takes away from the significant meaning of true allostery. There are even examples of allosteric behavior occuring in proteins when no net conformational change occurs, or when the allosteric effect derives from changes in dynamics rather than structure.